1. Types
2. Distribution
3. Asymmetry
4. Lateral
5. Synthesis
- Mitochondria
- Chlooplast
6. Functions
The LIPID MAPS (LIPID Metabolites And Pathways Strategy) defines lipid as a molecule insoluble in water and soluble in organic solvents. There are molecules in the cell fulfilling these properties, and they are referred to as biological lipids. There are a broad diversity of biological lipids, such as fatty acids, waxes, monoglycerides, diglycerides, triglycerides, phospholipids, sterols, terpenes, prenols, eicosanoids, fat soluble vitamins, and many others.
Cell lipids perform three main funtions: structural component of membranes, energy storing, signaling molecules. In this page, we will deal with membrane lipids.
membrane
models
The structural organization and properties of cell membranes are strongly influenced by lipids. Proteins and carbohydrates are the other components of membranes. The high diversity of lipid (more than one thousand types) and their organization capabilities (form bilayers) make lipid essential for both organization and function of membranes. Lipids account for about 50 % of the membrane weight, and there are about 5 millions of lipids per µm2. It is roughly estimated that 5 % of the cell genes are related to lipid metabolism.
Lipids strongly influence the physical features of membranes. The length and saturation of their fatty acid chains regulate fluidity and thickness of membranes. The uneven distribution of lipids between the two lipid hemilayers generates membrane asymmetry. The electrical charges located in the hydrophilic lipid heads contribute to the electrochemical gradient between both membrane surfaces, modulating the electrochemical membrane potential. Through lateral interactions, lipids can modulate the activity of membrane proteins. The lateral heterogeneity of cell membranes are thought to be caused by lateral lipid-lipid interactions, forming spatial and functional domains, such as the lipd rafts, which make a membrane multifuncional. For example, some proteins associate to certain membrane domains. Furthermore, lipids may work as second messengers, leaving membranes and diffusing to intracellular compartments to trigger cellular responses. The lipid composition of cell membranes is different depending on the cell compartment, and the lipid repertory is important to establish the compartment identity.
1. Types
A wide variety of lipids has been found in the eukaryotic cell membranes. They have been classified into three broad families of lipids: glycerophospholipids, sphingolipids and sterols.
Glycerophospholipids
Glycerophospholipids are the most abundant type of lipids in cell membranes, they account for more than 70 % of membrane lipids. They have 3 common components: two fatty acid chains, a glycerol, and a phosphoric acid (Figure 1). Other molecules bind to the phosphoric acid, increasing the lipid diversity. Fatty acid chains are 13 to 19 carbon atoms long. The fatty acid chains are the hydrophobic part (water phobia or water flee) of the molecule and makes the inner region of the membrane. Most carbon-carbon bonds are simple, which are referred to as saturated bonds. However, more than half of the fatty acids contain at least one double carbon-carbon bond, which is referred to as unsaturated. In each glycerophospholipid, the saturated chain is usually bond to the carbon 1 of the glycerol molecule, while the saturated one is linked to the carbon 2 of glycerol. Each double bond makes a permanent bend in the fatty acid chain and, although rotation of these chains is restricted, the increase of unsaturated fatty acids makes membranes more fluid because lipids are more separated to one another. The third carbon of glycerol is bond to a phosphoric acid, which works as a bridge to link a wide variety of molecular groups, such as choline, etanolamine, serine, inositol, and many others.

The main types of glycerophospholipids are phosphatidic acid (PA), phosphatidyl choline (PC), phosphatidyl ethanolamine (PE), phosphatidyl inositol (PI), caridolipin (CI), found in mitochondria, and lysophosphatidic acid (BMP/LSB), abundant in lysosomes and late endosomes/multivesicular bodies.
Glycosil phosphatidil inositol (GPI) is a type of glycosidated lipid (glycolipids). These lipids are ubiquitous in eukaryotic membranes. They are chemically linked to the carboxyl-terminus of proteins so that they function as an anchor that keeps proteins associated with membranes. GPI may also be found as free lipids in membranes. Their hydrophilic part contains phosphatidil inositol, glucosamine, three manoses, and ethanolamine phosphate. Proteins are linked to the phosphate group through an amide bond.
Glycerophospholipis are also part of blood serum lipoproteins and work as surfactant in the lung airways. The cell can store large amount of lipids as glycerolipids, which are similar to glycerophospholipids, but lack the phosphoric group and the hydrophilic head. Glycerolipids are neutral lipids stored as lipid droplets. Triacylglycerols, the more abundant glycerolipids, are made up of a molecule of glycerol linked to three fatty acid chains, each bound to one of the glycerol carbons. On the other hand, some glycerolipids may have carbohydrates directly linked to the glycerol molecule. These are the glyceroglycolipids, which are scarce in animals but frequent in plant and bacteria.
Sphingolipids
Sphingolipids contain a molecule of sphingosine, which is a nitrogenated alcohol with a long carbon chain. Sphingosine binds a fatty acid chain to form a molecule known as ceramide (Figure 2. See also ↗ ). Ceramide is an active biological molecule present in cell membranes, although in low concentration. The name ceramide actually encompasses a group of molecules differing in the length of the fatty acids. Diverse polar heads can be linked to ceramide, such as carbohydrates and phosphoric groups that give rise to a variety of sphingolipids. In this way, sphingolipids show a molecular organization similar to glycerophospholipids: two hydrophobic chains linked to a hydrophilic head. Sphingomyelin contains ceramide linked to a phosphoric group and one ethanolamine or one choline. Most glycolipids, which are those containing sugar in the hydrophilic domain, in animal cell membranes are sphingolipids (glycosphingolipids). Glycosphingolipds can be divided in two groups: glucosphingolipids and galactosphingolipids, bearing glucose or galactose as the first saccharide, respectively. Galactosphingolipids are abundant in the myelin of the nervous system, but also in the intestine, testis, and kidneys. Cerebrosides are those sphingolipids having just one saccharide.
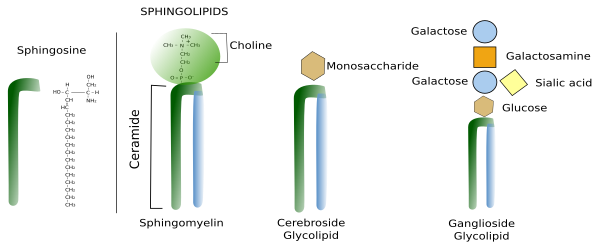
Sterols
Sterols are the third main group of membrane lipids. The molecule is made up of a hydroxyl or alcohol group in the carbon 3, four steroid carbonate rings and an aliphatic chain (Figure 3). Cholesterol is the most important sterol in animal cell membranes and the third most abundant type of lipid in the plasma membrane (from 10 % to 40 % of the total lipid content) in animal cells. There are some cells that show up to 50% of cholesterol in the plasma membranes, such as erythrocyte and myelin, and even higher in the fibrous cells of the eye lenses. However, it is scarce in membranes of organelles like endoplasmic reticulum (about 1 % to 5 %) and the Golgi apparatus (10 %).

Cholesterol is not present in plant cell membranes, in some unicellular eukaryotes, nor in bacteria. However, these cells show other types of sterols. Phytosterol is the most abundant sterol in plants, and ergosterol is in fungi. A large amount of cholesterol can be stored in the lipid droplets of animal cells as an energy reservoir.
Cholesterol is located among the other membrane lipids, with the polar region toward the membrane surface and the rest of the molecule between the fatty acids. Cholesterol, and the sterols, are essential for the integrity and functions of eukaryotic plasma membranes. They influence fluidity, stiffness and permeability of membranes, particularly the plasma membrane. Cholesterol is also involved in some metabolic processes, such as the synthesis of steroid hormones and bile acid. Moreover, it may modulate the activity of GPCR (G protein-coupled receptors), the signal transduction and vesicular trafficking. Together with sphingolipids, cholesterol contributes to create lateral membrane domains in the plasma membrane.
2. Lipid distribution in cell membranes
Many different types of lipids are distinctly distributed through the eukaryotic membranes (Figures 4 and 5), and sometimes showing large differences between each other. The structural and functional identity of membrane-bound organelles is determined by their molecules in their membranes, both lipids and proteins. For example, plasma membrane shows different lipid composition than those of the endoplasmic reticulum and Golgi complex. These differences are maintained even under a heavy flux of lipids between compartments carried by vesicles and transporters (Figure 5).
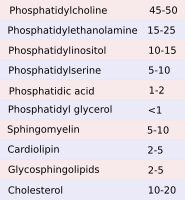
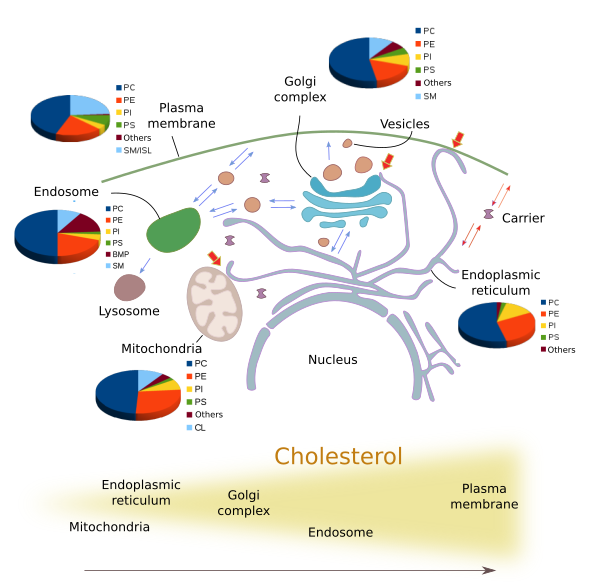
The amount and proportion of lipid species vary in the different cell membranes (Figure 5). Let's see some examples. All membranes contain phosphatidylcholine, but this lipid type is more abundant in the endoplasmic reticulum and in the inner mitochondrial membrane. The saturated fatty acid chains are more frequent in the plasma membrane, lowing fluidity and permeability. In post-Golgi membranes, i.e., plasma membrane and endosomes, the proportion of sphingolipids and cholesterol is much higher than in the endoplasmic reticulum and in the cis domain of the Golgi complex. The PI(4)P is abundant in the Golgi apparatus, and it has been used to identify this organelle. Mitochondria, besides other common lipids, contain cardiolipin as a characteristic lipid, synthesized by mitochondria themselves. It is interesting that during the maturation process of some organelles, such as endosomes, the lipid composition is gradually changed. The fosfatidic acid is abundant in the inner vesicles of the multivesicular bodies and in the late endosomes membranes, where it can be up to 15 % of the total lipids. On the other hand, cholesterol is more frequent as we move from the endoplasmic reticulum,to the Golgi apparatus, early endosomes and plasma membrane.
In a membrane, there is also a lateral segregation of lipid types, i. e. in the same hemilayer, constituing functional domains. Lipid-lipid and lipid-proteins interactions make transient stable associations that move laterally through the membrane. One of these associations, known as lipid rafts, are composed of sphyngolipids and cholesterol. Phospholipids with long fatty acid chains are found in the lipid rafts. It has been proposed that these domains may function as signalling platforms by recruiting a particular set of proteins. The phosphoinositides PI(3,4,5)P3 form another type of domain found in the phagocytosis membrane regions and in the front part of the plasma membrane of cells which are moving, whereas it is absent in the rear part. These membrane lateral heterogeneity may also be generated by local synthesis and degradation of lipids.
Diferential synthesis
The proportion of some lipid types is determined by the following they are synthesized and by the later delivery. The main source of lipids is the endoplasmic reticulum. Glycerophospholipids are synthesized in the endoplasmic reticulum, and they are more abundant in this organelle. Ceramide, which is the base for building sphingolipids, is also synthesized in the endoplasmic reticulum, but sphingolipids are assembled in the Golgi apparatus. Thus, sphingolipids are abundant in the Golgi and post-Golgi membranes (plasma membrane and endosomes). However, this is not a general rule. For example, cholesterol is synthesized in the endoplasmic reticulum, but the amount of cholesterol is higher in post-Golgi membranes because it is quickly transported by vesicular traffic and transporters from the endoplasmic reticulum to other organelles.
Tipid synthesis does not mean synthesis from scratch. Some lipids may be synthesized by chemical modifications of other lipids carried out by enzymes that remove and add chemical components to the molecular structure. These enzymes are specifically distributed in particular cell compartments. Thus, at the same time that a lipid is synthesized, another lipid disappears, both in the same membrane, and therefore the final lipid composition of this membrane changes. For example, maturation of some organelles, such as endosomes, involves a progressive modification of the lipid proportions, which is in part a consequence of local lipid modifications.
Selective transport
Lipids cannot freely travel through the cytosol because of their hydrophobic fatty acid chain. They can be transported between membranes as part of vesicle membranes, by long distance protein transporters, by protein transporters at the membrane contact sites, and very rarely by diffusion. Whatever the transport mechanism, it can select the lipid types to be carried, therefore changing the population of lipids in both the source and the target membrane.
The bulk transport of lipids in the cell is done by vesicles, that carry the lipids as components of their own membranes. This type of transport may show some selectivity when collecting the lipids to be included in the vesicle membrane. For example, the vesicles budding from the trans domain of the Golgi complex toward the plasma membrane and endosomes are enriched in sphingolipids and cholesterol, when compared with the concentration of these lipids in the Golgi complex membranes.
Although many lipids can be transported by vesicles, the delivery of lipids does not appear to solely depend on vesicular trafficking. In fact, after the inhibition of the vesicular trafficking, lipids continue to be transported between cellular compartments. There are proteins that work as lipid transporters. They take a lipid from the source membrane, protect the fatty acid chain from the aqueous environment of the cytosol, and insert the lipid in the target membrane. For example, CERT (ceramide transfer protein) transports ceramide from the endoplasmic reticulum membranes to the Golgi apparatus. There are many other transporters that transfer lipids between a variety of organelles, some of them non-connected by vesicular trafficking. For example, between the endoplasmic reticulum and the mitochondria. The transporters may be homotypic when they deliver the same lipid type, or heterotypic if they are able to transport more than one lipid type. It is thought that the lipid transport by transporters mainly happen in the membrane contact sites, where the distance between membranes of different compartments is really short (see below).
The transporter proteins are exchangers, and probably the transport work in both directions between the source and the target. And it is also possible that they can do both long and short distance transport. How they recognize a compartment as the source compartment may depend on the molecules that specifically recruit the transporters. How does a compartment get and maintain a particular lipid repertory? Several mechanisms have been suggested. For example, once the lipid is transferred to the target membrane, it is chemically modified, or it can be associated with resident molecules, or there is a thermodynamic kidnapping. Ceramide is quickly transformed into sphigomyelin or sphingolipid by chemical modifications. Cholesterol is trapped between the saturated fatty acids of the post-Golgi membranes (molecule-molecule interactions). Phosphatydil serine is retained in the cytosolic hemilayer of the plasma membrane by electric attraction (thermodynamic kidnapping).
Membrane contact sites
A at transmission electron microscopy, the membrane of some organelles can be observed very close to one another. These are hot spots for communication between the two compartments, largely for exchanging lipids. There are proteins placed between the two membranes that work as bridges, allowing the exchange of lipids. These proteins are transporters that protect the lipids from the aqueous cyitosolic environment. There are many observations of membrane contact sites involving the endoplasmic reticulum with other membranes, such as those of the Golgi apparatus, endosomes, and with the plasma membrane (Figure 6).
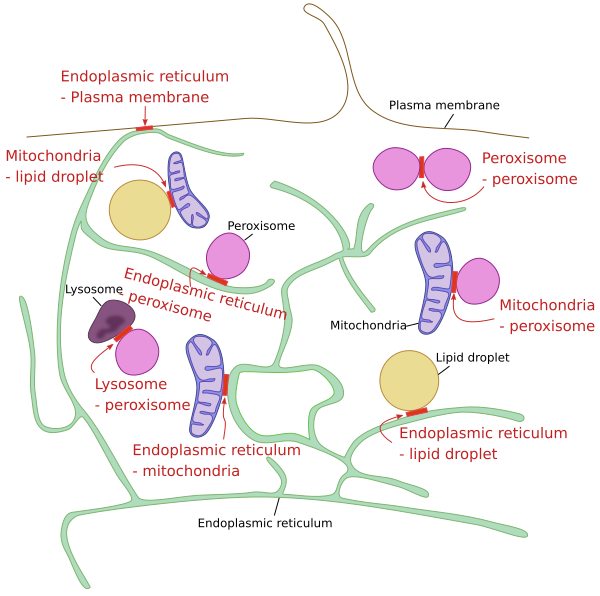
A part of the high concentration of cholesterol in the plasma membrane is generated by transport through these membrane contact sites. Cholesterol is co-transported with other lipids. The OSB (oxysterol binding protein) is an exchanger of cholesterol between the endoplasmic reticulum and the trans domain of the Golgi apparatus. PI(4)P is an abundant phosphoinositide in the trans domain of the Golgi apparatus. Thus, the gradient of PI(4)P, higher in the trans domain y lower in the endoplasmic reticulum, is used to transport cholesterol against the cholesterol concentration gradient, higher in the trans domain and lower in the endoplasmic reticulum. Once the PI(4)P is in the endoplasmic reticulum membrane, it is transformed in PI, so that the PI(4)P gradient can be maintained. In the trans domain membrane, cholesterol associates with sphingolipids to form more dense regions that are selected as exocytosis vesicles membranes, which are fused with the plasma membrane.
Membrane contact sites between lysosomes and peroxisomes are involved in the processing of cholesterol coming from the food. The extracellular cholesterol arrives to lysosomes by endocytosis, and is transferred from lysosomes to peroxisomes by membrane contact sites made by the two organelles. In peroxisomes, cholesterol enters metabolic pathways, such as the synthesis of bile acids.
3. Membrane asymmetry
Membrane asymmetry means a different lipid composition between the cytosolic hemilayer and the other hemilayer (Figure 7). The asymmetry is produced in the Golgi apparatus and other organelles. However, the endoplasmic reticulum show similar lipid composition in the two hemilayers. For lipids with a large hydrophilic component, it is difficult to jump to the other hemilayer because they can not cross the hydrophobic environment formed by the fatty acid chains. The movement between hemilayers is referred to as "flip-flop". However, other lipids with small polar heads, such as cholesterol, diacylglycerol, ceramide and protonated fatty acids, the movement between hemilayers is more common. Glycerophospholipids with large polar heads can cross the hydrophobic barrier with the help membrane proteins that works as trasnporters. There are three types: flippases, floppases and scramblases (Figure 8). These proteins use energy to exchange phosphoglycerolipids between the two hemilayers. Flipases trasnfer lipids toward the cytosolic hemilayer, floppases toward the luminal (or extracellular) hemilayer, and scramblases in both directions. They are actually three families of proteins, with members with differential affinity for particular lipid types. Once the asymmetry is generated in the membranes, it can be maintained by hydrophobic barrier that hinder the flip-flop movements. Sphigolipids, however, are not frequentely exchanged between hemilayers by these enzymes. They remain in the hemilayer where they are synthesized, the luminal hemilayer of the Golgi apparatus, that will be later the outer hemilayer of the plasma membrane. More than 80 % of sphingolipids of the plasma membrane are found in the outer hemilayer.


In the plasma membrane, the outer hemilayer contains a higher proportion of lipids with choline, such as phosphatidyl choline and sphingomyelin, whereas phosphatidyl ethanolamine, phosphatidyl inositol, and phosphatidyl serine are more abundant in the inner hemilayer. Diacilglycerol can change between hemilayers. When the environment is acid, such as in the lyosomes and late endosomes, some lipids can increase the probability of flip-flop movements. It may change the membrane asymmetry in these organelles. Although the endoplasmic reticulum membranes are "more" symmetric, there are some differences between the two hemilayers. For example, phosphatidyl serine is more abundant in the luminal hemilayer.
However, it seems that the asymmetry may change in the same compartment in different cell types. For instance, the proportion of phosphatidyl choline in the outer hemilayer of the plasma membrane is different when compare cell types, but also when compare the same cell type in different species. In human erythrocytes, about 76 % to 78 % of phosphatidyl choline in plasma membrane is found in the outer hemilayer, but it is only the 50 % in the outer hemilayer of the erythrocytes plasma membrane in mice.
4. Lateral distribution
There is an uneven distribution of lipids in the membranes of cell compartments, and also between the two hemilayers of most membrane. In addition, there are many evidences supporting a lateral heterogeneity of lipid distribution in the same hemilayer. It means that the lipids constituting a hemilayer are not randomly distributed, so that regions with different lipid composition are formed. These regions are regarded as membrane domains.
The membrane domains are generated by physicochemical lipid-lipid and lipid-protein interactions. Membrane recycling processes may be important too. It appears to be membrane domains with different features, such as stability, size, and major lipid types. That is, they are very dynamic entities. For example, they can change the size, from tenths to hundreds of µm, they can fuse and divide, and they appear and disappear in seconds to minutes.
The functional advantage of lateral membrane domains is that they may show differential physicochemical features that can favor some molecular processes. Some proteins "feel" more "comfortable" in some domains than in other regions of the membrane, and therefore may function more efficiently, accelerating the cellular process they are involved in. Furthermore, some molecular pathways need the concurrence of two proteins in the same place, and if these two proteins are more probably found in the same domain than outside, the probability that both proteins meet each other is higher. Finally, there are evidences that some viruses use particular membrane domains as platform for the infection, that is, enter the cell. The chemical environment of these domains facilitate cell recognizing and anchoring of the virus to the cell surface.
Several membrane domains have been described (Figure 9). Lipid rafts were proposed in 1997. They are small, tens to hundreds of nanometers, and very dynamic microdomains. They can grow, split, fuse and disappear easily. Lipid rafts consist of cholesterol and sphingolipids. Cholesterol is placed among the sphingolipid fatty acids, resulting altogether in a more compact molecular organization. Hence, lipid rafts are denser than the rest of the membrane, which is more fluid and mostly made up of phospholipids. These "rafts" would be able to move laterally, and proteins can enter and quit the lipid raft. Some of them would remain longer because of the molecular features of the lipid raft, which makes a suitable environment for the protein function, and may facilitate the physical proximity of proteins so that hey can interact more easily.

Caveolae are another type of membrane domain, mostly found in the plasma membrane. Cholesterol, sphingolipids and the protein caveolin are abundant in caveolae. This domain invaginates and usually form endocytic vesicles, carrying membrane receptors and other transmembrane proteins, that become trapped in caveolae because of their particular lipid composition. However, caveolae seem to have other functions, such as regulate the membrane composition and counteract mechanical stress.
Lipid-protein interactions can also result in membrane domains. Some transmembrane proteins can move laterally associated to a shell of lipids, which are attracted by electrochemical interactions. Thus, these lipids form a distinct membrane space around the protein.
It has been traditionally thought that both hemilayer of a membrane are largely independent when organizing their domains. However, there are evidences that one hemilayer may influence the lateral domains of the other. For instance, transmembrane proteins are present in both hemilayers, and therefore affect both hemilayers. The length of the lipid fatty acids, such as some sphingolipids, may be quite long (up to 24 carbons) and be inserted among the fatty acids of the other hemilayer. In this way, the distribution of lipids in one hemilayer affects the lipids of the other hemilayer. It has been proposed that when many lipids with long fatty acid chains gather in a small region, lipids with short fatty acid may be grouped in the other hemilayer to keep the membrane thickness homogeneous.
5. Synthesis
There are many enzymes involved in the synthesis of lipids. They are mostly found in the cytosol and associated to the endoplasmic reticulum and Golgi apparatus membranes. Enzymes participating in the cholesterol synthesis are found in other organelles too. It is of notice that lipid are not easily released by cells, unlike the hydrosoluble molecules. Thus, besides synthesis, cells need to have a wide repertory of enzymes to catabolize lipids. The uncontrolled accumulation of whatever lipid species may be toxic for the cell.
The three most abundant lipid types, glyceropholpholipids, sphingolipids and cholesterol, begins their assembling in the endoplasmic reticulum membranes.
Glycerophospholipids
L Glycerophospholipids are synthesized in the endoplasmic reticulum by enzymes found in its membranes, with the catalitic domain facing the cytosol (Figure 10). However, the synthesis of some glycerophospholipids are finished in the Golgi apparatus, and phosphatidyl ethanolamina can be synthesized in mitochondria. The synthesis process begins with a fatty acid chain transported through the cytosol and inserted in the cytosolic hemilayer of the endoplasmic reticulum membrane. The transporter is a protein knwon as lipid-binding protein. The fatty acid is activated by the addition of a Acetyl-CoA. The joining of the two activated fatty acids by a glycerol-3-phosphate is carried out by a acyl-tranferase enzyme, resulting in a phosphatidic acid. The phosphatidic acid looses a phosphate group to become diacyl glycerol. Phosphatidyl choline, phosphatidyl serine and phosphatidil inositol are synthesized by de addition of CDP-choline, CDP-serine or CDP-inositol, respectively. Phosphatidyl serine can be also synthesized from CDP-choline, phosphatidyl choline and from phosphatidyl ethanolamine. The enzymes in charge of these processes are concentrated in the endoplasmic reticulum, and are particularly abundant in the membrane contact sites formed between the endoplasmic reticulum and mitochondria.

The phosphatidic acid molecule is the base for building all glycerophospholipids, mostly done in the endoplasmic reticulum membranes, though a small proportion is also carried out in the outer mitochondrial membrane.
Glycerophospholipids may also be synthesized in almost each cell compartments by chemical modification of other preexisting glycerophospholipids. For instance, phosphatidyl ethanolamine gives phosphatidyl serine in the endoplasmic reticulum. Phosphatidyl serine, phosphatidyl choline and phosphatidyl ethanolamine may exchange their hydrophilic heads to became other lipid type. In this way, phosphatidyl choline may exchange the choline for a serine and becomes phosphatidyl serine. Other modifications may be chemical changes in the polar head. For example, phosphatidyl ethanolamine can be generated from phosphatidyl serine. The complete hydrophilic head can be removed to get diacyl glycerol again. Another example is the synthesis of phosphatidic acid in the multivesicular bodies by modifications of local lipids. Sometimes, the exchange of polar heads may be between glycerophospholipids and sphingolipids. Thus, phosphatidyl choline may donate the phospho-choline to a sphingolipid, resulting in a sphingomyelin in the Golgi apparatus.
Phosphoinositides are an illustrative example of how chemical modifications lead to new lipid types, together with new functions. Phosphoinositides can be phosphorylated in 3 sites of their polar head, that gives to mono-, di-, and tri-phosphrylated molecules. In addition, the different combination of phosphorylated sites in mono- and di-phosphorylations results in even more lipid types. The specific phosphatases and phosphorylases found in each compartment determines the type of phosphoinositide in their membranes. On the other hand, the phosphoinositide molecular base is assembled from CDP-diacyl glycerol, not from diacyl glycerol.
Sphingolipids
Ceramide is the molecular frame to build sphingolipids. Ceramide is synthesized in the endoplasmic reticulum from one amino acid, mostly serine, and acyl-coenzime A, giving to di-hydro-sphingosine. Linking a fatty acid results in ceramide (Figure 11). Then, ceramide is transported to the Golgi apparatus to assemble the sphingolipids. This delivery is accomplished by two mechanisms: vesicles and ceramide transporters (CERT). However, the enzyme that add galactose to galactosphingolipids is found in the endoplasmic reticulum.

In the cis domain of the Golgi apparatus, ceramide may follow two pathways: synthesis of sphingomyelin by the addition of a phosphoric group and a choline, or synthesis of glucosphingolipids by the addition of a glucose. Sphyngomyelins are the most abundant sphingolipids in membranes and are essential for the cells. Without sphingomyelins the cell can not live. The addition of the phosphoric group and the choline produces both a sphingomyelin and a diacyl glycerol. Glucosphingolipids are transported to the trans domain of the Golgi apparatus and become glycosphingolipids by the addition of more saccharides, mediated by glycosyl transferases. Animals that cannot synthesize glucosphingolipids are not viable. However, cell cultures with cells lacking this sphingolipids can proliferate. The interaction of the phosphoinositide PI(4)P with some enzymes in the Golgi apparatus is needed for the synthesis of sphingolipids.
Cholesterol
The cholesterol present in the cell may come from two sources: synthesized by the cell itself or coming from outside the cell (food or released by other cells). The synthesis of cholesterol in the cell is a long metabolic pathway with many enzymes involved found in different organelles, although most of them are associated to the endoplasmic reticulum membranes. The hidroxyglutaryl reductase and those enzymes participating in the last steps of cholesterol synthesis are found in the endoplasmic reticulum (Figure 12). Cholesterol is quickly transported from the endoplasmic reticulum to other cell membranes. The amount of cholesterol synthesized by a cell is regulated by the cholesterol concentration within the cell, so that excessive cholesterol removes the enzymes involved in the cholesterol synthesis. The hydroxymethylgluraryl reductase is the main enzyme involved in the detection of intracellular cholesterol levels. The excess of cholesterol leads to the cholesterol esterification and storing as lipid droplets.

Cholesterol coming from the food is transported from the intestine to the liver by the portal system. In the liver, cholesterol is packaged into low density lipoproteins (LDL) and shipped to the rest of the body through the vascular system. LDL enters the cell by receptor-mediated endocytosis, reaches the endosomal compartment, and lysosomes, where LDL is degraded. From lysosomes, cholesterol is distributed to other cell compartments. It is estimitated that about the 80 % of an animal cell (excepting neurons) is got by receptor-mediated endocytosis.
Tissues can get cholesterol from both inner and external sources. However, the nervous tissue can only get cholesterol by local synthesis. It is a consequence of the hematoencephalic barrier.
5.1. Mitochondria
The lipid composition of the inner mitochondrial membrane is essential for the activity of the oxidative phosphorylation chain and ATP synthase. Mitochondrial membranes show lipids found in other cell compartments, such as phosphatidyl choline, phosphatidyl ethanolamine, phosphatidyl inositol and phosphatidyl serine. However, they also have exclusive lipids: phosphatidyl glycerol and cardiolipin. Mitochondrial membranes contain very low proportion of sphyngolipids and cholesterol.
The function of cholesterol in the plasma membrane, where it balances the hydrophobicity and fluidity, is done by cardiolipin in the inner mitochondrial membrane. The hydrophobicity (impermeability) is an important feature of the inner mitochondrial membrane because it affects the proton gradient that is generated for ATP synthesis. In addition, cardiolipin provides a proper environment for the proteins of the respiratory chain. For instance, the lack of caridiolipin leads to molecular mal-formations and desestabilization of some respiratory molecular complexes. In addition, cardiolipin is involved in the importation of proteins from the cytosol (some of these proteins are synthesized in the cytosolic ribosomes).
Mmitochondria divide and fuse, which means split and fuse membranes. Cardiolipin, phosphatidic acid and phosphatidyl enthanolamine are needed for these processes.
Phosphatidyl choline and phosphatidyl serine are synthesized in the endoplasmic reticulum, and they must be imported by mitochondria. Phosphatidyl ethanolamine can be formed in mitochondria by modification of phosphotidyl serine, or be imported from the endoplasmic reticulum. Independently of where synthesis place, mitochondrial lipids need to pass from one membrane to the other, and this seems to happen at physical contact sites made by these membranes. Membrane contact sites between the endoplasmic reticulum and the mitochondria also allow the swap of lipids between these two organelles.
5.2. Chloroplast
Chloroplasts are the main center for fatty acid production in plant cells. These fatty acids are exported to the endoplasmic reticulum, where most membrane lipids are synthesized. Diacyl glycerol, which is also synthesized in the endoplasmic reticulum, returns to chloroplasts for the assembling of galactosil glycerolipids. As it happens with mitochondria, the communication between the endoplasmic reticulum and chloroplasts is necessary to keep the lipid composition of both organelles.
Chloroplasts show a distinct lipid composition. Galactosyl glycolipids are both synthesized and found only in chloroplasts. Galactolipids are essential for chloroplasts, particularly for thylakoid membranes, where optimal conditions have to be set for the optimal function of the photosynthetic proteins. Galactolipids may be up to 25 % to 50 % of the total lipid content of the thylakoidal membrane, and up to 60 % of the inner and outer membranes of the chloroplast. Phosphatidyl glycerol is the only phospholipid synthesized in the chloroplast, necessary for the function of the photosystem II of the photosynthetic machinery. The lipid composition of cyanobacteria membranes is similar to chloroplasts, and it makes sense because both share the same common ancestor.
6. Functions
Membrane structure
The molecular features of lipids lead them to spontaneously form bilaminated membranes, such as cell membranes. Phosphatidyl choline is the main responsible for this feature because it is the most abundant lipid in membranes (excepting in Drosophila!). However, the other lipid types can also form membranes. The lipid composition of a membrane, including the polar head diversity and length of fatty acids, determines the fluidity, thickness, bending and electric charge of that membrane. Lipids influence the activity of membrane proteins and contribute to the anchoring and association of proteins to membranes.
Phosphatidyl choline is about 50 % of the lipid content of the eukaryotic membranes. They often have one saturated fatty acid chain, while the other is unsaturated. Spatially, they show a cylindrical form, which makes them influence membrane fluidity and work as good structural components. Besides structural organization, membrane lipids are involved in other membrane features. Thus, sphingolipids, unlike phosphatidyl choline, have two saturated and longer fatty acid chains, which lead to more dense and thicker membranes. Cholesterol may increase or decreases the fluidity of membranes depending on its concentration, fluidity and temperature. In general, a higher cholesterol concentration, more saturated fatty acids, and higher concentration of sphingolipids make cell membranes more dense (less fluid) and thicker. It leads to a more impermeable membrane, such as the plasma membrane. The inner membranes are more fluid and thinner than the plasma membrane.
Phosphatidic acid and phosphatidyl ethanolamine show a conic tridimensional conformation. This shape favors membrane curvatures and membrane fusion processes. A membrane bend leads to both a concave hemilayer and a convexe hemilayer. Conic shaped lipids with small hydorphylic heads promote convexe hemilayers, while those with large polar heads help to form concave hemilayers. This rule is accomplished in artificial membranes. However, cell membrane curvatures are mostly induced by proteins, although conic lipids help to form and estabilize these bendings.
Many cytosolic proteins are transiently recruited to membranes by lipid-protein interactions. The lateral lipid domains of membranes associate with different sets of proteins, leading to different functional domains in the same membrane. In addition, some lipids, such as phosphatidyl inositol, are the anchoring molecules to get proteins permanently associated to membranes, usually to the non-cytosolic hemilayer.
The distribution of glycerophospholipids with net negative charge in their polar heads (anionic lipids) are more abundant in the cytosolic hemilayer of the plasma membrane. Thus, there is negative net electric charge in this surface, which allow two functions: contribute to the membrane potential and form an electrical environment suitable for the recruitment and function of some cytosolic proteins, as well as for the functioning of the cytosolic domains of transmembrane proteins. Phosphatidyl inositol and phosphatidyl serine are anionic lipids, and some sphingolipids are cationic lipids (positive net charge). However, most lipids do not show net electric charge. Membrane asymmetry, particularly in the plasma membrane, generates a negative charge in the inner surface and a positive charge in the outer one. It has been demonstrated that in an ion free medium, the membrane asymmetry is enough for generating a membrane potential. The cytosolic and extracellular domains of transmembrane proteins are affected by this electric environment. For instance, the probability for the opening of an ionic channel is influenced by the membrane potential. Thus, the variation in the proportion of some lipids in a hemilayer change the membrane potential, and therefore the functionality of some ion channels. As mentioned above, the electric environment of the cytosolic membrane surface boosts the protein recruitment. For example, K-Ras, Src and Rac1 are polycationic proteins attracted by anionic lipids. The negative charge of the cytosolic surface depends on the cell compartment. The cytosolic hemilayer of the endoplasmic reticulum is nearly neutral, good for promoting biochemical reactions.
The effect of lipids in membrane fluidity and permeability is explained in this page: ↗
Signaling
Lipids influence and participate in cell signalling. It may be either by making proper environments that favor signaling pathways or by acting as signalling molecules. Lipid rafts are environments where some molecular processes are favored because proteins may interact with their partners more easily or because the chemical environment benefits the protein activity.
Some lipids are signals in the signalling pathways. Disorganization of membrane asymmetry of the plasma membrane is a signal telling that something wrong is going on in the cell. For example, apoptotic cells expose phosphatidyl serine in the outer hemilayer. This lipid is a signal "saying" to macrophages, "eat me". The asymmetry breakage is also a signal during blood clotting. Some virus coated with membrane place phosphatidyl serine and phosphatidyl ethanolamine in the outer hemilayer to be more easily endocyted by phagocytosis or macropinocytosis.
There are more examples. The phosphatidyl inositol PI(4,5)P2 can be found in the inner hemilayer of the plasma membrane. Some phopholipases catabolize this phosphoiniositide and gives two molecules. One of them is IP3, which is freed into the cytosol, travels to the endoplasmic reticulum to stimulate the calcium release from this organelle. Phosphatidyl choline is a source of arachidonic acid, which is transformed in prostaglandins.
Vesicular trafficking
Membrane lipids are essential for vesicular trafficking. During endocytosis (clathrin dependent endocytosis, phagocytosis and macropinocytosis), lipids are active players.
Clathrin-mediated endocytosis needs the phosphoinositide PI(4,5)P2 during the first stages of vesicle formation: nucleation, cargo selection and coat assembling, but it should disappear before later phases like vesicle excision and getting rid of the protein coat. Clathrin vesicles are initiated in membrane regions rich in PI(4,5)P2 because this phosphoinositide recruits the proteins needed for vesicle nucleation, such as GTPases and the BAR-type proteins amphiphysin and endophilin for membrane bending. Other proteins involved in later steps, like dynamine and the adaptor proteins AP-2, are also recruited by PI(4,5)P2. However, for vesicle excision and the latter removing of the vesicle coat, a strong decrease in PI(4.5)P2 is needed in the membrane vesicle. This reduction is carried out by phosphatases that are recruited during the vesicle formation. It is interesting that beside proteins, removing PI(4,5)P2 helps in the vesicle excision process.
Phosphatidyl ethanolamine is a major player during the formation of autophagosomes by recruiting soluble adaptor proteins. It also interacts with membrane proteins, influencing their activity. PI(4,5)P2 also participates in phagocytosis by the association with actin filaments, and probably facilitating actin polimerization. In the same way that during clathrin vesicle formation, PI(4,5)P2 is removed before the later stages of phagocytosis before the phagosome formation. PI(4,5)P2 is actually transformed into PI(3,4,5)P3, which may recruit the myosin motor protein. The maturation of phagosomes is similar to the maturation of endosomes. However, phagosomes need to generate reactive oxygen substances for killing pathogens by oxidation. These enzymes are recruited by the phosphoinositide PI(3)P.
PI(4)P is abundant in the Golgi apparatus. This phosphoinositide participates in the synthesis of sphyngolipids and recruits AP-1 adaptor protein, which regulates the vesicular trafficking.
PI(3)P is involved in the maturation of endosomes. It can be formed from PI(3,4,5)P3, PI(3,4)P2, o by kinases that modified other phospholipids, such as the Vps34 kinase. Proteins participating in the endosome maturation associate first to PI(3)P. As endosome maturation progresses, PI(3)P is degraded. Several chemical reactions remove PI(3)P from endosome membranes. One of them is the conversion PI(3)P into PI(3,5)P2, which is a marker of late endosomes. In addition, in the multivesicular bodies, PI(3)P is removed from the endosome membrane included in the membrane of the inner vesicles. During endosome maturation, the lysophosphatidic acid is generated. This lipid is important for the formation of the inner vesicles of the multivesicular bodies.
-
Bibliography ↷
-
Bibliography
Alberts A, Johnson A, Lewis J, Raff M, Roberts K, Walter P. 2007. Molecular Biology of the Cell. 5th editon. Garlan Science. ISBN: 9780815341055.
Becker WM, Kleinsmith LJ, Hardin J, Raasch J. 2003. The world of the cell. 6th. San Francisco: Benjamin Cummings. ISBN-10: 0321716027 .
Bohdanowicz M, Grinstein S. 2013. Role of phospholipids in endocytosis, phagocytosis, and macropinocytosis. Physiological reviews. 93: 69-106.
Carquin M, D’Auria L, Pollet H, Bongarzone ER, X Tyteca D. 2016. Recent progress on lipid lateral heterogeneity in plasma membranes: from rafts to submicrometric domains. Progress in lipid research. 62:1-24.
Edidin M. 2003. Lipids on the frontier: a century of cell-membrane bilayers. Nature reviews in molecular and cell biology. 4: 414-418.
Funai K, Summers SA, Rutter J. 2020. Reign in the membrane: How common lipids govern mitochondrial function. Current opinion in cell biology. 63: 162-173.
Drin, G. 2014. Topological regulation of lipid balance in cells. Annual review of biochemistry. 83: 51-77.
Hammond GRV, Burke JE. 2020. Novel roles of phosphoinositides in signaling, lipid transport, and disease. Current opinion in cell biology. 3: 57-67.
Holthuis JCM, Menon AK. 2014. Lipid landscapes and pipelines in membrane homeostasis. Nature. 510: 48-57.
Ikonen E, Zhou X. 2021. Cholesterol transport between cellular membranes: balancing act between interconnected lipid fluxes. Developmental cell. 56: 1430-1436.
Lev S. 2010. Non-vesicular lipid transport by lipid-transfer proteins and beyond. Nature reviews in molecular and cell biology. 11: 739-750.
Nicolson GL. 2014. The Fluid—Mosaic Model of Membrane Structure: Still relevant to understanding the structure, function and dynamics of biological membranes after more than 40years. Biochimica et Biophysica Acta (BBA)-Biomembranes. 1838(6): 1451-1466.
Pollard TD, Earnshaw WC, Lippincott-Schwartz J. 2007. Cell biology. 2th edition. Saunders Elsevier Inc. ISBN: 978-1-4160-2255-8.
Quazi F, S. Molday RS. 2011. Lipid transport by mammalian ABC proteins. Essays in biochemistry. 50, 265–290.
Schenkel LG, Bakovic M. 2014. International journal of cell biology. Article ID 709828, 13 pages.
Schrader M, Godinho LF, Costello JL, Islinger M. 2015. The different facets of organelle interplay—an overview of organelle interactions. Frontiers in cell biology 254: 151-213.
Subczynski WK, Pasenkiewicz-Gierula M, Widomska J, Mainali L, Raguz M. (2017). High cholesterol/low cholesterol: effects in biological membranes: a review. Cell biochemstry and biohysic. 75: 369-385.
Thelen AM, Zoncu R. 2013. Emerging roles for the lysosome in lipid metabolism. Trends in cell biology. 27: 833-850.
Tidhar R, Futerman AH. 2013. The complexity of sphingolipid biosynthesis in the endoplasmic reticulum. Biochimica et biophysica acta. 1833: 2511–2518.
Vance JE. 2014. Phospholipid synthesis and transport in mammalian cells. Traffic, 2014:709828. doi: 10.1155/2014/709828.
van Meer, de Kroon AIPM. 2011. Lipid map of the mammalian cell. Journal of cell biology. 124: 5-8.
-