1. Structure
2. Instability
3. MAPs
4. Motor proteins
5. MTOCs
6. Functions
- Organization
- Cilia / flagela
Microtubules are a component of the cytoskeleton involved in the internal organization of eukaryote cells. They carry out many functions. Thus, they contribute to the spatial organization of organelles, work as tracks for vesicular trafficking, are needed during cell division by forming the mitotic spindle, participate in cell movement, contribute to cell polarization in some cell types, and are an essential component of cilia and flagella. Microtubules have been found in all eukaryotic cells so far; therefore, they were present in LECA (last eukaryotic common ancestor). Many prokaryotic cells contain at least a gene that codes for a tubulin-homologue protein, and it is involved in cell division.
1. Structure
Microtubules are long and relatively stiff tubules (Figures 1 and 3). They are the thickest elements of the cytoskeleton, about 25 nm in diameter, and from 1 to 100 µm in length. Their walls are made up of many dimers of globular proteins: α- and β-tubulin (Figure 2). The tubulin dimers did are evolutionary conserved. Despite this, in humans, there are 9 genes for α-tubulin and another 9 for &alpha-tubulin. The core of tubulins is similar, but the C-terminal, which protrudes from the microtubule surface, may be variable and affected by chemical modifications. The content of a particular tubulin subtype influences the stability, dynamic and mechanical properties of the microtubule, as well as the interaction with the microtubule-associated proteins (MAPs). It is interesting that several subtypes of tubulin may co-localize in the same microtubule. Some particular microtubules, such as those forming cilia and flagella, can be distinguished by a distinct composition of tubulin subtypes. For instance, TubB1 (a subtype of β-tubulin) is expressed in blood platelets, TubB3 is mostly found in neurons, and TubB4 is found in the axoneme of cilia and flagella. There are other less frequent tubulin subtypes, such as δ, ε, and ζ, that contribute to a small proportion of microtubules in centrioles, cilia and flagella. These three tubulin subtypes are found in many eukaryotic lineages, but they seem to be lost in other lineages.



Pairs of α- and β-tubulin line up to form long strands of dimers known as protofilaments. A typical microtubule is made up of 13 protofilaments attached laterally to one another, although 15 or 16 protofilamentes may be present in some microtubules. The joining between the protofilament 1 and 13 differs from the others, and it is observed as a seam. In this seam, the association of the β-tubulins of one protofilament with the α-tubulins of the other is weak. The joining between the other protofilaments is stronger because it is done between the α-tubulins of one protofilament and the α-tubulins of the adjoining protofilament. The same happens with the β-tubulins. Thus, the microtubule is more unstable because of the seam. It is also interesting that the joining between tubulin dimers in one protofilament is stronger than the adhesion between adjacent protofilaments. Microtubules are not spiral lines of tubulin dimers but are straight rows of dimers, the protofilaments, associated laterally, and each of them grows and shortens independently. In general, the walls of protofilaments are described as solid, but they show some passages and some cracks that allow the exchange of water and other molecules between the interior of the microtubule and the cytosol. The tubulin dimers are chemically modified. They can be acetylated, phosphorylated, sumoylated, detyrosinated, and polyglutamylated. Most of these modifications occur in the microtubule. Acetylation is done in the interior of the microtubule, but many others affect the C-terminus of tubulin found on the microtubule surface. In addition, the C-terminus is one of the key elements that interact with MAPs, and it is thought that chemical modifications of the C-terminus may affect the interaction with MAPs. The chemical modifications of tubulin are largely observed in the more stable microtubules, such as neurons, axonemes of cilia and flagella, and centrioles. For instance, acetylation is a key element in identifying stable microtubules. Glutamylation is abundant in the microtubules of centrioles, neurons, cilia, and flagella. Detyrosination is abundant in microtubules found in the front domain of cells during movement and in the mitotic spindle.
Within a protofilament, all α- and β-tubulin dimers are oriented in the same way (Figure 13). There is always the α-tubulin of the first dimer at one end of the protofilament and the β-tubulin of the last dimer at the other end. All protofilaments in a microtubule are oriented in the same way. It means that microtubules are polarized structures. The end formed by α-tubulins is known as the minus end of the microtubule, and the end formed by β-tubulins is known as the plus end. Microtubules are dynamic structural elements, and periods where new tubulin dimers are added alternate with others where tubulin dimers are removed; that is, polymerization and depolymerization, respectively. New tubulin dimers are mainly added to the plus end (α-tubulin), where the growth of the microtubule usually happens, although depolymerization also occurs. In the minus end, depolymerization prevails over polymerization.
2. Dynamic instability
Microtubules are highly dynamic structures, continuously undergoing polymerization and depolymerization, and mainly happening at the plus end (Figure 4). There is a steady exchange of tubulin dimers between the cytosol and microtubules. In a typical fibroblast, half of the available tubulin is free in the cytosol, and the other half is part of microtubules. This behavior is quite different from Intermediate filaments, in which the subunits are largely in the polymerized state.
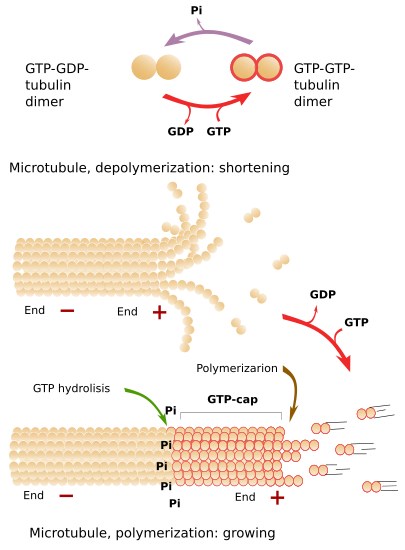
The addition of new tubulin dimers to the plus end makes the microtubule grows in length (Figure 4). The growth is stopped from time to time, and growth periods alternate with shrinkage periods. Depolymerization is sometimes so strong that the complete microtubule may disappear. However, a new polymerization (growing) period is more frequent. The alternation between polymerization and depolymerization of microtubules is known as dynamic instability.
Free tubulin dimers are linked to two GTP molecules, one to the α-tubulin and the other to the β-tubulin. Some time later, after the joining of a tubulin dimer to the plus end of a microtubule, the GTP of the β-tubulin is hydrolyzed to ADP. This process is stimulated by the next polymerized dimers, which increases the GTPase activity. If the rate of adding GTP-GTP-tubulin dimers to the plus end is faster than the hydrolysis rate (GTP-GDP), there will always be a group of tubulin dimers with GTP-GTP in the plus end, which is known as GTP-cap. GTP-cap can be for 10 dimers long and stabilizes the plus end of the microtubule. Under this situation, the polymerization is favor and the microtubule grows. However, the polymerization speed depends on the environment condition at the plus end tip. On the other hand, if the addition rate of new GTP-GTP-tubulin dimers is low, the hydrolysis rate may overcome the polymerization speed. This means that there are GTP-GDP-tubulin dimers at the plus end, which makes protofilaments weakly adhere to one another. In this situation, massive depolymerization starts. If the plus end is stabilized by external elements, the microtubule grows again (Figure 4). The GTP-ADP-tubulin dimers released to the cytosol during depolymerization quickly phosphorylated to GTP-GTP-tubulin dimers, which are ready to join a microtubule plus end. It is of note that the GTP hydrolysis is not intended to get a more stable microbutule, but to make it weak and easily disassembled. This can be proved when the GTP is changed by a GTP analogous that is very difficult to hydrolyze. The result is that microtubules polymerize as usual, but they do not depolymerize. Polymerization, although regulated, is an spontaneous process.
Why to waste so much energy in an inefficient process because of the dynamic instability? Cells are always changing in both, behavior and morphology, and microtubules have to be adapted in length and orientation to reach different places of the cytoplasm, or to form new structures, such as the mitotic spindle. The transition from growing to microtubule destruction is known as catastrophe, whereas the change from depolymerization to growing is called rescue. During microtubule catastrophe, the protofilaments curve outward because it is the most favorable disposition of GTP-GDP dimers, although it means easier depolymerization.
An interesting behavior is observed in microtubules when both ends are free. Under this situation, microtubules are shortened through the minus end and enlarged by the plus end. The final result is that the microtubule looks like it is moving in the plus-end direction, and actually the microtubule changes its position. This mechanism is called treadmilling (walking), and it is more often observed in plant cells.
3. MAPs
Microtubules are associated with a variety of proteins, which control polymerization, depolymerization, and the spatial organization within the cell. The microtubule surface is coated with the C-teminus of the tubulin dimers giving an overall negative charge to the structure. The amino acid chains that form the microtubule surface interact with the microtubule-associated proteins (MAPs). Although initially, only the proteins that co-sedimented with microtubules were called MAPs, there are many other proteins that interact with microtubules. "Naked" microtubules are relatively unstable structures, and they can do nothing, so MAPs actually rule the microtubule functions. Many MAPs are evolutionary ancient, but some are only found in some species.
MAPs can be classified according to their effect on microtubules. Thus, there are those that stabilize or make unstable (including those that disorganize) microtubules, those that protect the microtubule ends, and those that establish bridges between microtubules and other cell structures. Some of them may do more than one function. Stabilizing MAPs promote microbutule polymerization or minimize depolymerization. For instance, Tau, MAP2, MAP4, and STOP protiens, stabilize microtubules under low temperature. Doblecortin is important for nervous tissue microtubules, and EMAPs in cancer. Desestabilizing proteins function by different mechanisms. Some of them join to free tubulin dimers, such as statmine, others stabilize the plus end, such as the depolymerizing kinesins, and there are some that break down microtubules, such as catanin, spastin, and fidgentin. γ-tubulin rings protect the microtubule minus end and prevent depolymerization and polymerization. MAPs that establish bridges with other microtubules attach to the lateral wall of microtubules. MAP65/Ase1/PRC1 are proteins that join microtubules to one another in an antiparallel manner, which is important for structures like the mitotic spindle. Some MAPs link microtubules with other cellular structures, which can be other cytoskeleton components. The interaction of microtubules and actin filaments is needed during cytokinesis and during the establishing of cell polarity. The connection between microbutules and membranes and organelles can be done by specific MAPs different from motor proteins. Finally, there are enzymes associated with microtubules, such as some enzymes of the glycolytic pathway.
MAPs can also be classified according to what part of the microtubule they are attached to. Tau, MAP2 and MAP4 attach to the microtubule wall. At the plus end, not only one, but several types of proteins can be concurrently attached and form a scaffold. γ-tubulin and the CAMPSAPs proteins join the minus end.
Some substances affecting microtubule function have been used as medicines or as toxins. For instance, colchicine inhibits the microtubule polymerization, whereas taxol does the opposite and inhibits microtubule depolymerization.
4. Motor proteins
Motor proteins are a type of protein that can be attached to the microtubular wall and move either toward the plus or toward the minus end. Dynein and kinesin are the two motor protein families. Dynein moves toward the minus end of the microtubule, and kinesin toward the plus end. Structurally, both protein types comprise a globular domain that binds ATP and interacts with the microtubule, and a tail domain that recognizes and binds a specific cargo to be transported. ATP hydrolysis in the globular domain changes the molecular conformation and makes the protein moves along the microtubule, dragging the cargo.
Dyneins are divided into two subfamilies: those found as part of the axoneme of cilia and flagella, and those found in the cytosol. In the axoneme, dynein slides one pair of peripheral microtubules over the adjoining one, eliciting the movement of the cilium and flagellum. However, the transport of molecules along the long axis of the cilium is carried out by cytosolic dyneins (subtype 2). The cytosolic dyneins in the cytoplasm (subtype 1) are in charge of intracellular transport, as well as other functions.
There are 45 genes in the human genome for kinesins, that can be divided into 14 families. All of them show a globular domain, a stalk, and a tail domain. Those kinesins that do not link a cargo through the tail domain cannot move along microtubules because the free tail inhibits the globular domain (the motor unit). There are intermediary proteins that mediate the joining of the kinesin and the cargo.
Some motor protein subtypes prefer microtubules with particular chemical modifications. For instance, kinesin-1 more often joins acetylated or detyrosinated microtubules. This preference may be mediated by MAPs.
5. MTOCs
The concentration of cytosolic tubulin dimers is not high enough to spontaneously polymerizes into new microtubules. In the lab, microtubules can polymerize at 20 µM of tubulin dimers, which is actually the average concentration in the cell cytosol. However, in the cell, due to the features of the molecular milieu, there are molecular structures known as microtubule organizing centers (MTOCs), that are needed to rule microtubule formation. MTOCs are the structures where new microtubules are formed and where their minus ends are used to be anchored.
MOTCs contain γ-globulin and other associated proteins. There are two types of MOTCs. The small MTOCs contain two γ-tubulin molecules and two associated proteins. The large MTOCs are made up of several γ-tubulin molecules that organize helicoidally to form a ring-like structure. In vitro, the capacity to form a new microtubule is 150 times higher in larger MTOCs than in smaller ones. The γ-tubulin rings are formed by the lateral association of other smaller complexes. In yeast, only small complexes are observed.
The γ-tubulin rings are circular structures that work as templates to build microtubules. Beside nucleation, these rings also protect the minus ends of microtubules. There are γ-tubulin rings in the cytosol, but they need to be activated by additional proteins to start microtubule nucleation. it is estimated that only about 1 % of the total γ-tubulin rings are activated in a cell. This activated pool concentrates in the MTOCs. The recruitment of γ-tubulin rings to MTOCs (centrosomes, Golgi apparatus, and mitochondria) depends on proteins, such as CM1. TPX2 and XMAP25, in collaboration with the γ-tubulin rings, make possible the nucleation process. In many eukaryotes, it is possible to nucleate a new microtubule on the surface of an existent one. For that, besides γ-tubulin proteins, the augmin protein is involved. This type of nucleation is observed during mitosis, in order to increase the amount of kinetochoric microtubules.
The centrosome is the major MTOC in animal cells (Figure 5). It is the main responsible for the number, localization and spatial organization of microtubules. In the 1980s, Bovery and others described the centrosome associated with the mitotic spindle poles in both normal and cancer cells. About a century later, the centrioles of the centrosomes were observed with the electron microscope. In most animal cells, during the G1 and G0 phases of the cell cycle, there is only one centrosome per cell found near the nucleus. However, megakaryocytes contains multiple centrosomes, and muscle fibers lack centrosomes. The centrosome consists of two components: a couple of orthogonally oriented centrioles and a surrounding pericentriolar material. Each centriole is a cylindrical structure with a wall made up of 9 triplets of microtubules. A typical centriole is 0.5 µm long and 0.2 µm wide.
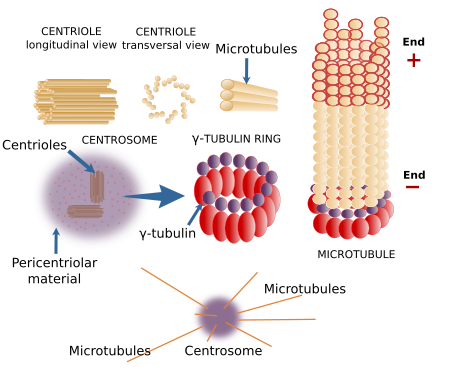
There are many proteins in the pericentriolar material, including many activated γ-tubulin rings. That is why the centrosome is a major MTOC. Pericentrin is a molecule that retains γ-tubulin rings in this compartment. Pericentriolar material seams to be organized in domains, and the Drosophila centrosome has been divided in 5 regions. Centrioles, however, do not participate in the formation of microtubules nor in their spatial orientation, excepting the distal and subdistal centriolar appendages, which are structures attached to the mature centriole that can nucleate microtubules. The function of centrioles is still unknown. For example, plant cells lack centrioles, but they can segregate chromosomes, divide into two new cells, and organize their microtubules without any problem. It has been suggested that centrioles keep the pericentriolar material concentrated in one point of the cytoplasm. Centrioles are similar to basal bodies, structures located in the basal part of the cilia and flagella, from where microtubules in the cilia and flagella are nucleated´.
The centrosome is also essential for the cell cycle regulation because there are many proteins in the pericentriolar material involved in the advance of the cell cycle and because it is needed for the formation of the mitotic spindle. For example, duplication of the centrosome before mitosis of animal cells is necessary to produce two "healthy" new cells. This duplication means that two poles are formed by the two centrosomes available to nucleate the bipolar mitotic spindle. In this regard, the supernumerary centrosomes that are common in cancer cells form multipolar mitotic spindles, which leads to aneuploidies and to genome instability and more aggressive cancer. However, centrosomes are not indispensable for mitotic spindle formation. For instance, plant cells lack centrosomes, and centrosomes removed by microsurgery do not prevent the formation of mitotic spindles in animal cultured cells. Other sources of centrosomes, such as chromosomes, can lead to functional mitotic spindles.
The Golgi apparatus is the second major MTOC in the animal cells, both for microbutule nucleation and microtubule anchoring. The Golgi apparatus cisterns are able to nucleate microtubules, which help to keep cisterns in organized and cohesive stacks. In addition, microtubules nucleated from the Golgi apparatus contributes to establishing cell polarity because the number of microtubules is higher where the Golgi apparatus is found. For instance, more than half of the microtubules are nucleated in the Golgi apparatus of retinal pigmented cells. The reorientation of microtubules toward the cellular front during cell movement seems to depend more on the Golgi apparatus than on the centrosome. In addition, the Golgi apparatus rules the microtubule nucleation in muscle cells and pancreas cells. AKAP450, a pericentrin-like protein, recruits γ-tubulin to the Golgi cisterns membrane. It is interesting that the Golgi apparatus can fetch the minus ends of free microtubules and stabilize them in a γ-tubulin rings-independent manner, but this is mediated by MAPs.
TThere are other cellular structures where microtubules can be nucleated. In many cell types, like neurons and epithelial cells, the microtubule organization is not radial, but microtubules are organized in an antiparallel manner. For that, they have their minus ends anchored and protected in different cytosolic structures. Blepharoplasts are molecular complexes found in plant cells and in some animal cells that can nucleate microtubules, and sometimes they can also form centrioles and centrosomes. Plant cells lack centrioles and do not form typical centrosomes, but they have γ-tubulin rings associated with the nuclear envelope, blepharoplasts, and are scattered through the cytoplasm. Plant cells nucleate microtubules more frequently in the peripheral cytoplasm. The multiciliated cells of the respiratory ducts are able to synthesize many basal bodies (similar to centrioles) very quickly from cytosolic complexes known as deuterosomes. The main MTOC in yeasts is the polar body, which is inserted into the nuclear envelope. There are other structures that nucleate microtubules, such as chromosomes, which can form a mitotic spindle without centrosomes.
6. Function
Microtubules perform many functions. The type of function is mostly regulated by MAPs. MAPs stabilize, generate forces, and connect microtubules with other cellular structures, including other microtubules. In addition, there is a tubulin dimer code, which is set by the expression of different tubulin isoforms and particular post-translational modifications.
Intracellular organization
Microtubules are classified into two types: stable microtubules, found in cilia and flagella, and dynamic microtubules, found in the cytosol. The usual organization of microtubules in animal cells is radial, with the minus ends converging in the cetrosome and the plus ends more or less perpendicular to the cell surface. However, there are many other organizations (Figure 6). Thus, prismatic and cuboidal epithelial cells show microtubules parallel to the basal-apical axis, with the plus end toward the apical part and the minus end toward the basal domain. In plant cells, many microtubules are arranged below the cell membrane and oriented more or less perpendicular to the major axis of the cell.

Cytosolic microtubules, besides their role in the mitotic spindle formation and chromosome segregation, are also involved in the internal movement of organelles such as mitochondria, lysosomes, pigment inclusions, and lipid droplets. They are also needed for vesicular trafficking. When cells in culture are observed at light microscopy, organelles show an alternation between quick movement and quiet periods. This behavior, known as saltatory movement, occurs when organelles are moving along the microtubules. In plant cells, however, the internal movements of organelles are mostly ruled by actin filaments. Plant cell microtubules found underneath the plasma membrane seams to participate in setting the orientation of the cellulose fibers during cell wall synthesis, which influences cell growth. The movement of organelles is essential for all eukaryotic cells, but it is clearly needed for neurons to transport organelles along the axons until the axon terminals, journeys sometimes as long as several centimeters. The inner cellular organization based on microtubule distribution also contributes to establishing polarized cells.
The movement of organelles along microtubules is actually the dragging process carried out by the motor proteins. Motor proteins are also involved in setting the organelle shape and the final location of large organelles, such as the Golgi complex and the endoplasmic reticulum. When colchicine, a depolymerizing substance, is added to the cell, both organelles collapse and break down into small vesicles that get dispersed through the cytoplasm. When the drug is removed, and microtubules can assemble again, both organelles are formed with the typical morphology and in their normal places. It indicates that in the membrane of these organelles, there are proteins that are recognized by the motor proteins.
Cilia and flagella
Cilia and flagella are cellular structures that outgrowth from the cell surface, contain microtubules, and are delimited by the plasma membrane. Cells use cilia and flagella to move the surrounding liquid, to move themselves if they are free cells, or as sensory structures. Cilia are shorter than flagella and more numerous, and their movements propel the liquid parallel to the cell surface. Flagella move the surrounding liquid perpendicularly to the cell surface.
Cilia and flagella are complex structures made up of more than 250 different proteins (Figures 7 and 8). Although the molecular structure is similar, they can have different molecular composition. Both have a central structure of microtubules known as axoneme, separated from the extracelular environment by the plasma membrane. The axoneme is composed of 9 outer pairs of microtubules, plus a central pair of microtubules. This organization is written down as 9x2+2, which is interlocked by inner molecular linking. The axoneme grows from the basal body, which has 9 triplets of microtubules (9x3+0), the same organization as centrioles. The outer pairs of microtubules of the axoneme are connected to each other by nexin proteins, whereas protein spokes connect the outer pairs with the central pair. The motor protein dynein is located between the outer pairs. Dynein is involved in the movement of cilia and flagella, by sliding a pair of microtubules over the other, leading to the bending of the whole structure.

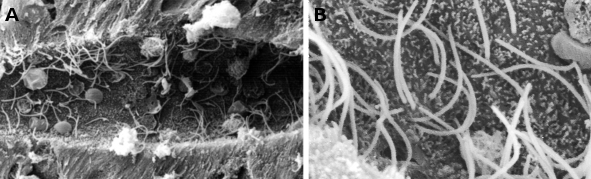
Some types of cilia are known as primary cilia. Primary cilia lack the central pair of microtubules, cannot move, are scarce, and sometimes appear alone in the cells. At least one cilium is present in most animal cells studied so far. Primary cilia bear in their membranes many receptors and ionic channels, so a sensory role has been suggested. Nowadays, primary and common cilia are regarded as sensory structures, since both show receptors in their membranes.
-
Bibliografía ↷
-
Bibliografía
Farache D, Emorine L, Haren L, Merdes A. 2018. Assemby and regulation of γ-tubulin complexes. Open biology. 8: 170266.
Gadahar S, Bodakuntla S, Natarajan K, Janke C. 2017. The tubulin code at a glance. Journal of cell science. 130: 1347-1353.
Goodson HV, Jonasson EM. 2018. Microtubules and microtubule-associated proteins. Cold Spring Harbor perspective in biology. 10:a022608.
Ohi R, Zanic M. 2016. Ahead of the curve: new insights into microtubule dynamics. F1000Research. 5:314.
-